Sebastien Hamel, Miguel Morales, Kyle Caspersen
The predictive capability of QMD simulations allows us to determine the properties of materials subject to planetary interiors conditions. The equation-of-state (EOS) of the planetary materials, specifically the pressure as a function of density, temperature, and composition, are required in order to close the set of hydrostatic equations used in planetary models [1,2,3].
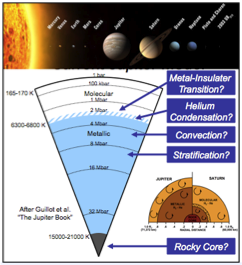
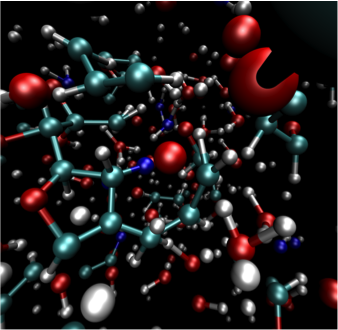
[1] D. C. Swift, J. H. Eggert, D. G. Hicks, S. Hamel, K. Caspersen, E. Schwegler, G. W. Collins, N. Nettelmann and G. J. Ackland Mass-Radius Relationships for Exoplanets. Astro. Phys. J. 744, 59 (2012)
[2] R. G. Kraus, S. T. Stewart, D. C. Swift, C. A. Bolme, R. F. Smith, S. Hamel, B. D. Hammel, D. K. Spaulding, D. G. Hicks, J. H. Eggert, G. W. Collins Shock vaporization of silica and the thermodynamics of planetary impact events. J. Geophys. Research – Planets 117, E09009 (2012)
[3] N. Nettelmann, K. Wang, J.J. Fortney, S. Hamel, S. Yellamilli, M. Bethkenhagen, R. Redmer, Uranus evolution models with simple thermal boundary layers. Icarus Vol. 275, pages 107-116 (2016)
[4] Morales, MA; Schwegler, E; Ceperley, D; Pierleoni, C; Hamel, S; Caspersen, K. Phase separation in hydrogen-helium mixtures at Mbar pressures. P. Natl. Acad. Sci. USA, 106, 1324-1329, (2009)
[5] S. Hamel, Miguel Morales and Eric Schwegler, Signature of helium segregation in hydrogen-helium mixtures. Phys. Rev. B 84, 165110 (2011)
[6] Stephanie Brygoo, Marius Millot, Paul Loubeyre, Amy E Lazicki, Sebastien Hamel, Tingting Qi, Peter M Celliers, Federica Coppari, Jon H Eggert, Dayne E Fratanduono, Damien G Hicks, J Ryan Rygg, Raymond F Smith, Damian C Swift, Gilbert W Collins, Raymond Jeanloz, Analysis of laser shock experiments on precompressed samples using a quartz reference and application to warm dense hydrogen and helium. Journal of Applied Physics 118 (19), 195901 (2015)
[7] R. Chau, S. Hamel, and W. J. Nellis Chemical Processes in the Deep Interior of Uranus. Nature Communications, 2, (2011)
[8] Ping Y, Coppari F, Hicks DG, Yaakobi B, Fratanduono DE, Hamel S, Eggert JH, Rygg JR, Smith RF, Swift DC, Braun DG, Boehly TR, Collins GW, Solid iron compressed up to 560 GPa. Phys Rev Lett. 111 065501 (2013)
[9] Marius Millot, Sebastien Hamel, J. Ryan Rygg, Peter M. Celliers, Gilbert W. Collins, Federica Coppari, Dayne E. Fratanduono, Raymond Jeanloz, Damian C. Swift & Jon H. Eggert, Experimental evidence for superionic water ice using shock compression. Nature Physics 14, pages 297–302 (2018)
[11] M. French; S. Hamel and R. Redmer Dynamical Screening and Ionic Conductivity in Water from Ab Initio Simulations. Phys. Rev. Lett., 107, 185901 (2011)
[12] M. Bethkenhagen, E. R. Meyer, S. Hamel, N. Nettelmann, M. French, L. Scheibe, C. Ticknor, L. A. Collins, J. D. Kress, J. J. Fortney, and R. Redmer, Planetary Ices and the Linear Mixing Approximation. The Astrophysical Journal Vol. 848, page 67 (2017)
[13] Mandy Bethkenhagen, Daniel Cebulla, Ronald Redmer, and Sebastien Hamel,
Superionic Phases of the 1:1 Water–Ammonia Mixture. The Journal of Physical Chemistry A 119 (42), 10582-10588 (2015)